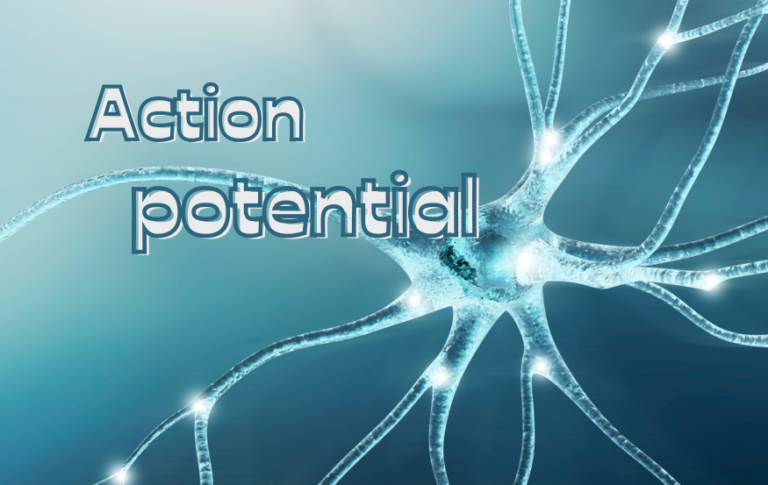
How can neurons generate electrical signals?
Some time ago, we wrote that neurons communicate using electrical and chemical signals. We also mentioned that neurons generate electrical impulses at the level of their soma, that is, their cell body, which then propagate to their terminal buttons.
In this blog post, we explain how these electrical impulses, called “action potentials”, are produced.
First, it’s important to know that the membrane potential of a neuron, which corresponds to the difference in electrical potential on either side of its membrane, can be measured with a very small electrode (called a microelectrode) introduced into the neuron’s cytosol (the liquid part around the cell’s nucleus) and a reference electrode placed outside the neuron (see Figure 1).
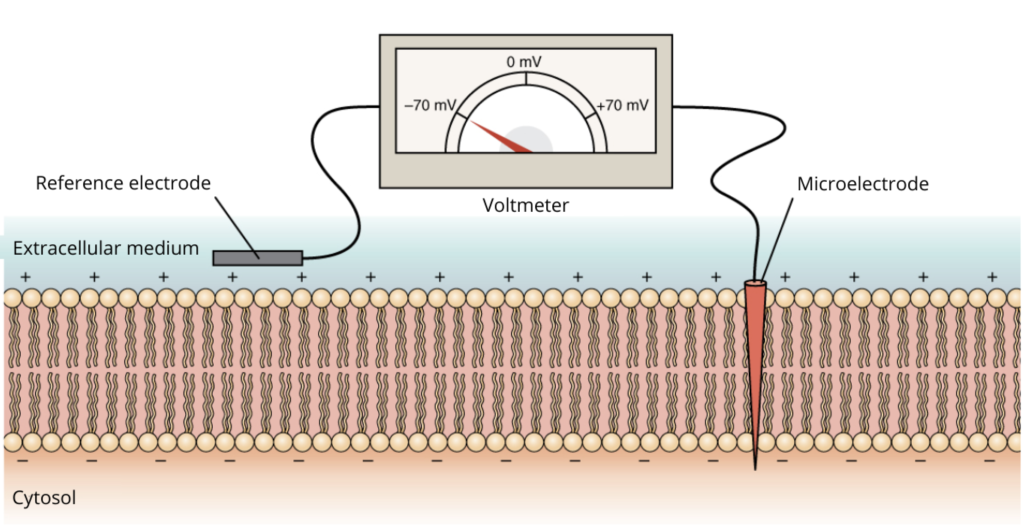
Figure 1. Illustration of a voltmeter measuring the membrane potential of a neuron, using a microelectrode inserted into the membrane and a reference electrode in the extracellular medium. The membrane potential of this neuron is equivalent to -70 mV, as indicated by the voltmeter. There is thus a potential difference of 70 mV between the internal and external parts of the membrane, the internal part being more negative. Adapted from the original image (1220 Resting membrane potential) from OpenStax under CC BY 4.0 license.
The membrane potential of the neuron varies depending on whether it is at rest or active. Changes in potential are made possible by the numerous channels that cross the membrane of the neuron, forming pores that allow the passage of certain molecules (ions), such as sodium (Na+) and potassium (K+). Some of these channels are like small pumps that exchange the Na+ ions present inside the cell with K+ ions present outside the cell (see Figure 2). These pumps ensure that, at rest, the concentration of Na+ is higher in the extracellular medium (i.e., outside the neuron), while the concentration of K+ is higher in the intracellular medium. This gives the inner part of the resting neuron’s membrane a negative electrical charge compared to the extracellular medium. This is called the “resting potential”. The resting potential varies depending on the type of neuron, but generally falls between -40 and -90 mV.
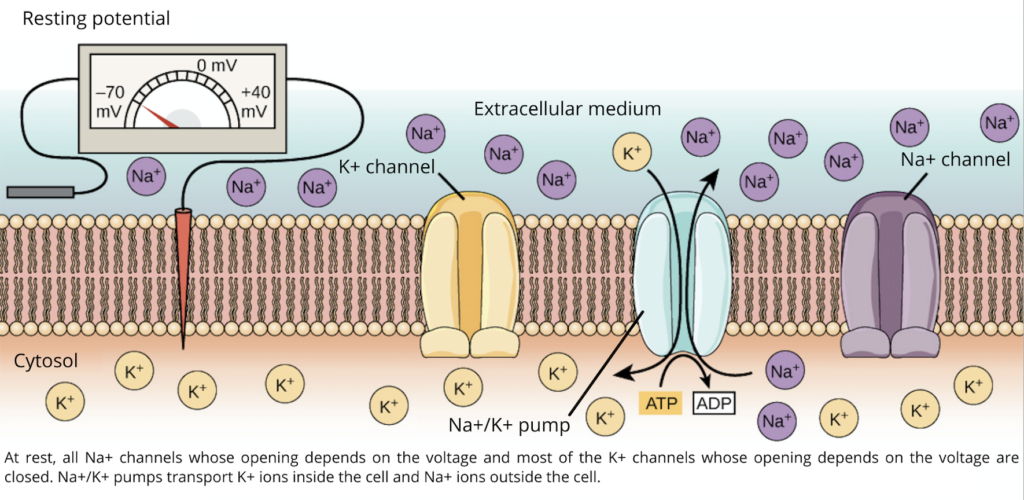
Figure 2. Illustration of the movements of Na+ and K+ ions when the neuron is at rest. Adapted from the original image (Ion channel activity before during and after polarization) by Robert Bear and David Rintoul under CC BY 4.0 license.
An action potential occurs when this state is rapidly and temporarily reversed, i.e., when the inside of the membrane becomes positively charged relative to the outside of the neuron for a very short period. Chemical signals from other neurons cause changes in membrane potential that can lead to an action potential: when neurotransmitters bind to receptors located on the dendrites of a neuron, the opening or closing of ion channels modifies the membrane potential as well as its propensity to generate an action potential. The action potential can be recorded using an oscilloscope. This instrument allows the visualization of a plot representing the membrane potential of a neuron over time (see Figure 3). The plot shows that the action potential is very fast, approximately 2 milliseconds!
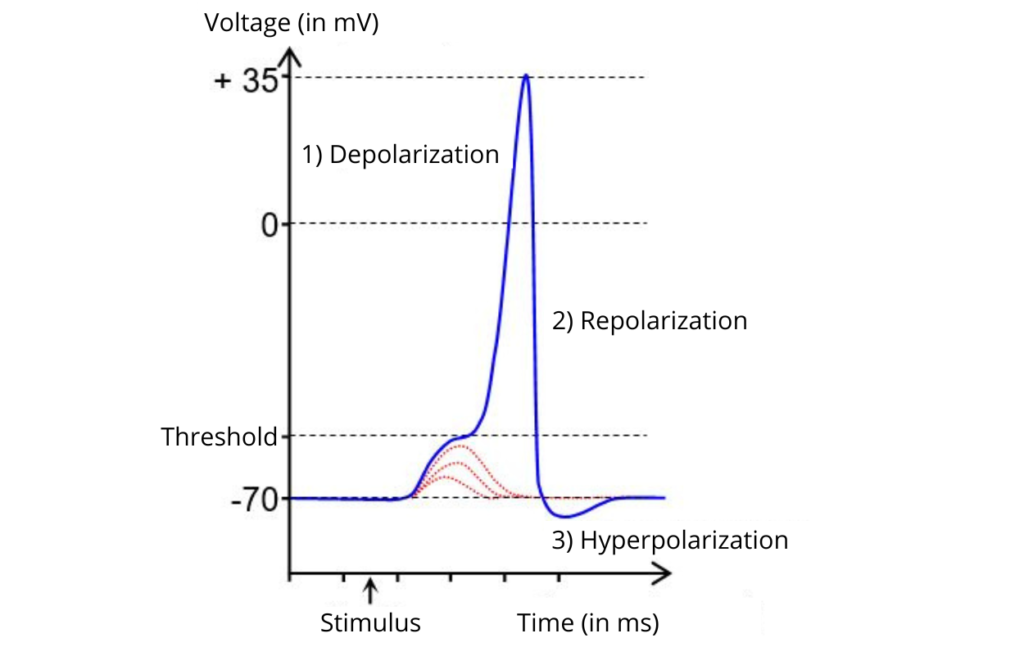
Figure 3. Action potential (PA threshold action potential), image adapted from Groh64 under CC BY-SA 4.0 license. The red lines illustrate changes in membrane potential that do not lead to the firing of an action potential, as the threshold is not reached.
The action potential has several phases:
1. Depolarization.
Membrane depolarization is caused by the entry of Na+ ions into the neuron, which pass through the channels located in the neuronal membrane. The opening of these channels is usually controlled by neurotransmitters released by other neurons (see our post on synaptic transmission). When Na+ ions enter the neuron, the cytosol becomes less negative. If the membrane potential reaches a certain threshold (see Figure 3), an action potential is triggered: voltage-sensitive sodium channels open and rapidly allow more Na+ ions to enter the neuron (see Figure 4). The change in membrane potential continues until it reaches a peak at nearly +40 mV. This is when the reversal of potential is observed, i.e., the potential inside the membrane is more positive than in the extracellular medium. Voltage-sensitive sodium channels are open for about 1 millisecond.
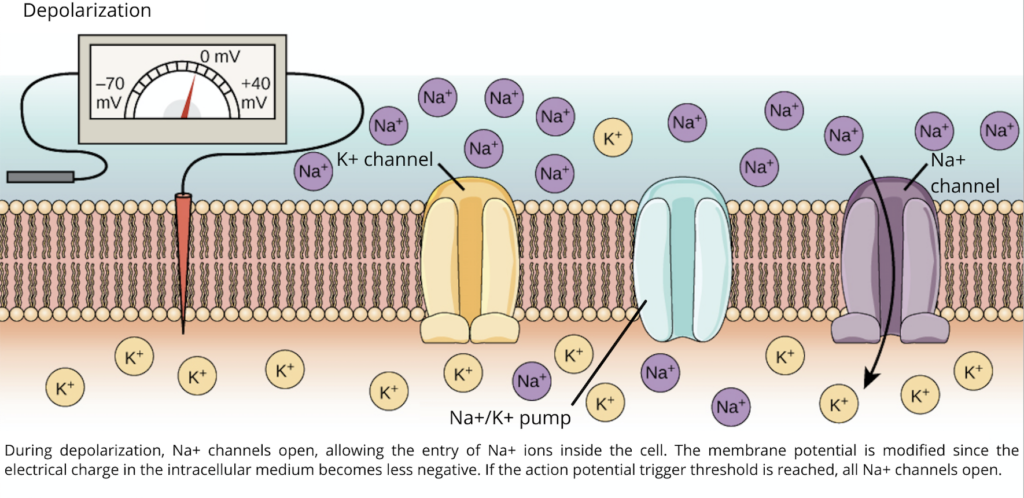
Figure 4. Illustration of the movements of Na+ ions during the depolarization phase. Adapted from the original image (Ion channel activity before during and after polarization) by Robert Bear and David Rintoul under CC BY 4.0 license.
2. Repolarization.
The sodium channels close, and their reopening cannot take place until the membrane potential has returned to a negative value near the threshold for triggering an action potential. This closing of the channels is called inactivation. As soon as the voltage-sensitive sodium channels are inactivated, the potassium channels located in the membrane open. This opening results in the rapid movement of K+ ions from inside the cell to the extracellular medium (see Figure 5). The membrane potential then becomes negative again, which corresponds to the repolarization phase (see Figure 3).
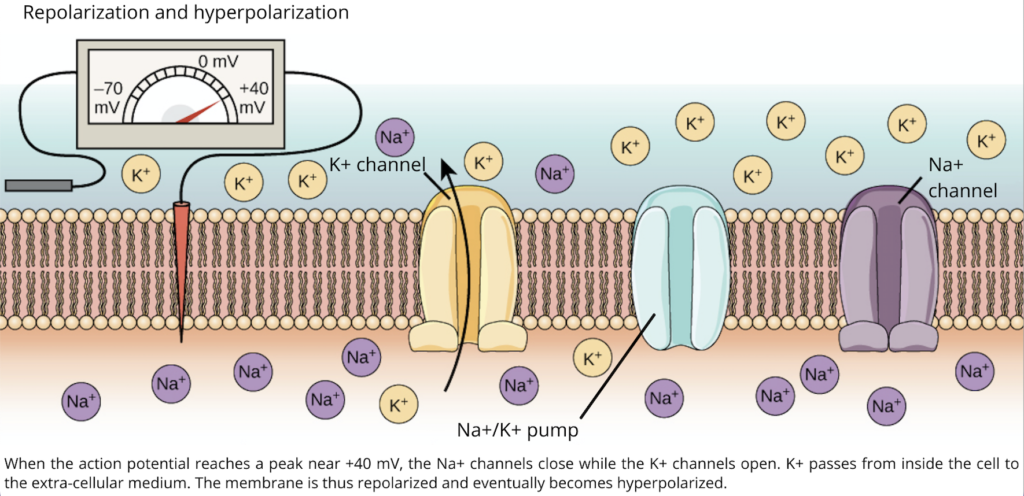
Figure 5. Illustration of the movements of K+ ions during the phases of repolarization and hyperpolarization. Adapted from the original image (Ion channel activity before during and after polarization) by Robert Bear and David Rintoul under CC BY 4.0 license.
3. Hyperpolarization.
The hyperpolarization phase occurs while the potassium channels are still open and the sodium channels are closed. Since potassium channels increase the permeability of the membrane to K+, but are less permeable to Na+, the membrane potential becomes more negative compared to the resting potential. This is called hyperpolarization (see Figure 3), which ends when the potassium channels close. The membrane of the neuron then returns to its resting potential.
In our lab, we do not measure the membrane potential of neurons, as this type of research is conducted using brain slices from animals or cell cultures. However, we use neuroimaging techniques to measure or modify the electrical activity of neurons. For example, transcranial magnetic stimulation (TMS) can locally induce action potentials in the neurons located under the coil! Stay tuned, we will come back to this in an upcoming publication on the mechanisms of TMS!
Here are the books that were used to write this blog post and that you could consult for more information on action potentials:
Purves, D., Augustine, G.J., Fitzpatrick, D., Hall, W.C., LaMantia, A.S., & White, L.E. (Eds). (2015). Neuroscience (5th ed.). Sinauer Associates.
Bear, M.F., Connors, B.W., & Paradiso, M.A. (2007). Neuroscience: Exploring the brain (3rd ed.). Lippincott Williams & Wilkins Publishers.